The human society is facing tremendous challenges with respect to depleting fossil energy and increasing energy demands. In order to solve these issues, the construction of efficient and clean energy conversion and storage devices, such as wind turbines, photovoltaic cells, and lithium-ion (secondary) battery, is essential. One-dimensional (1D) nanostructured materials such as nanofibers (NFs), nanowires (NWs), nanotubes (NTs), and nanorods (NRs) [3] have attracted extensive attention due to their unique physical and chemical characteristics. Among these 1D nanostructured materials, continuous NFs have been the focus of studies because of their unique properties compared to other nanostructured materials in the application of energy devices. Until now, numerous methods such as template-assisted synthesis, chemical vapour deposition (CVD), self-assembly, wet chemical synthesis, and electrospinning have been applied to the preparation for nano fibrous materials. Compared to other methods, electrospinning has the merits of simplicity, high efficiency, low cost, and high reproducibility. Electrospinning was first developed as a patent to produce continuous fibers in 1934. Since then, much attention has been focusing on this technique and its applications. As a versatile method, electrospinning can be applied to the fabrication of one-dimensional (1D) nanomaterials of polymers, inorganic materials, and composites with diameters ranging from tens of nano meters to several micro meters. Particularly, compared to 1D materials synthesized with other methods, the NFs prepared by electrospinning have larger specific surface areas, higher aspect ratio, and better pore interconnectivity, which are favorable for energy conversion and storage. In addition, electrospun NFs with various morphologies and structures such as core-shell, hollow, yarn, and porous structures can be obtained by modifying process parameters including applied voltage, feed rate, collector type, tip-to-collector distance, nozzle design, and calcination treatment.
Nanofibers are able to form a highly porous mesh and their large surface-to-volume ratio improves performance for many applications. Porous structures with their high surface areas have found applications in many different areas. Nanofibers, with their large surface-to-volume ratio, have the potential for use in various applications where high porosity is desirable. A porous structure made out of nanofibers is a dynamic system where the pore size and shape can change, unlike conventional rigid porous structures. Nanofibers can also be linked to form a rigid structure if required. Perhaps the most versatile process for producing nanofibers with relatively high productivity is electrospinning. Porous, nanofiber meshes made by electrospinning have been identified for use in numerous applications.
There are several methods of producing nanofibers, from high-volume production methods such as melt fibrillation1, island-in-sea2, and gas jet3 techniques, to highly precise methods like nanolithography4, 5 and self-assembly6, 7, 8, 9. However, their usefulness is limited by combinations of restricted material ranges, possible fiber assembly, cost, and production rate. Here, electrospinning has an advantage with its comparative low cost and relatively high production rate. Micron size yarns consisting of nanofibers can be produced at a rate of 70 m/min, and different assemblies can be formed.
Almost any soluble polymer with sufficiently high molecular weight can be electrospun. Nanofibers made of natural polymers, polymer blends, nanoparticle- or drug-impregnated polymers, and ceramic precursors have been successfully demonstrated. Different fiber morphologies have also been shown, such as beaded, ribbon, porous, and core-shell fibers10.
As with conventional fiber-spinning process, the solution typically passes through a spinneret during electrospinning, although there are a few exceptions. But instead of using air or mechanical devices to create the extrusion force, a high voltage is applied such that the particles within the solution are charged, thereby creating a repulsive force. At a critical voltage, the repulsive force overcomes the surface tension of the solution and a jet erupts from the tip of the spinneret. Unlike conventional spinning, the jet is only stable near the tip of the spinneret, after which the jet is subject to bending instability11. As the charged jet accelerates toward regions of lower potential, the solvent evaporates while the entanglements of the polymer chains prevent the jet from breaking up. This results in fiber formation. Generally, a grounded plate is used to collect the fibers.
Electrospinning was first patented in the US in 190212; however, the process was largely forgotten until the 1990s. With interest in the field of nanoscience and nanotechnology, researchers began new investigations of nanofiber production using electrospinning13. This effort has grown, with over 200 universities and research institutes worldwide studying various aspects of the electrospinning process and the fibers it produces. The number of patents for processes and applications based on electrospinning has also grown in recent years. Startups such as eSpin Technologies, NanoTechnics, and KATO Tech are just some of the companies seeking to reap the unique advantages offered by electrospinning, while companies such as Donaldson Company and Freudenberg have been using electrospun fibers in their air filtration products for the last two decades.
The ability to form porous fibers through electrospinning means that the surface area of the fiber mesh can be increased tremendously. Phase separation is proposed as the main mechanism behind the formation of porous fibers. When more volatile solvents are used, solvent-rich regions begin to form during electrospinning that transform into pores14. Another method of producing porous nanofibers is the spinning of a blend of two different polymers. One of the polymers is removed after fiber formation by dissolution in a solvent in which the other polymer is insoluble15.
Since stretching of the solution arises from repulsive charges, the electrospinning jet path is very chaotic and only nonwoven meshes are produced using a typical setup. Nevertheless, more ordered assemblies that allow the porosity of the mesh to be controlled have been produced through clever manipulation of the setup and solution composition. Several methods have been developed that yield aligned fibers with various degrees of order16, 17, 18, 19 and fiber directions20, 21 for two- and three-dimensional assemblies22, 23, 24, 25, 26 (Fig. 6). Such assemblies are usually achieved through control of the electric field between the tip of the spinneret and the collector, use of a dynamic collector such as a rotating mandrel, or a combination of both. Li et al.20 used a pair of parallel conducting electrodes to create an electric field such that the electrospun fibers are preferentially aligned across the gap in between the electrodes. Boland et al.16 used a rotating drum at a speed of 1000 rpm to collect aligned fibers. To fabricate a tubular scaffold, electrospun fibers can be deposited on a rotating tube and the deposited fiber layer subsequently extracted from the tube. Fiber alignment can be controlled using auxiliary electrodes to create an electric field profile that influences the flight of the electrospinning jet
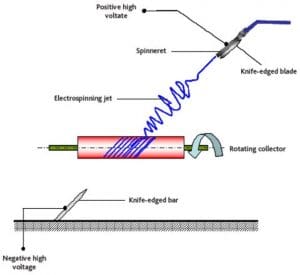
Controlling fiber alignment on a tubular scaffold through mechanical rotation and modification of the electric field.
With such versatility, electrospun fibers are being explored for use in many different applications. Currently, most tests use nonwoven fiber meshes made out of smooth fibers. Ceramic nanofibers derived from nonwoven electrospun fiber meshes have opened up new areas of opportunities. Besides nonwoven meshes, testing of other fibrous assemblies for potential applications has been limited. Nevertheless, the versatility of electrospun fibers can be seen in the established results and on-going research in major areas like healthcare, biotechnology and environmental engineering, defense and security, and energy storage and generation.
Healthcare applications:
Current medical practice is based almost entirely on treatment regimes. However, it is envisaged that medicine in the future will be based heavily on early detection and prevention before disease manifestation. Together with nanotechnology, new treatment modalities will emerge that will significantly reduce medical costs.
With recent developments in electrospinning, both synthetic and natural polymers can be produced as nanofibers with diameters ranging from tens to hundreds of nanometers with controlled morphology and function. The potential of these electrospun nanofibers in human healthcare applications is promising, for example in tissue/organ repair and regeneration, as vectors to deliver drugs and therapeutics, as biocompatible and biodegradable medical implant devices, in medical diagnostics and instrumentation, as protective fabrics against environmental and infectious agents in hospitals and general surroundings, and in cosmetic and dental applications.
Tissue/organ repair and regeneration are new avenues for potential treatment, circumventing the need for donor tissues and organs in transplantation and reconstructive surgery. In this approach, a scaffold is usually required that can be fabricated from either natural or synthetic polymers by many processing techniques including electrospinning and phase separation.
The biocompatibility of the scaffold is usually tested ex vivo by culturing organ-specific cells on the scaffold and monitoring cell growth and proliferation. An animal model is used to study the biocompatibility of the scaffold in a biological system before the scaffold is introduced into patients for tissue-regeneration applications.
Nanofiber scaffolds are well suited to tissue engineering as the scaffold can be fabricated and shaped to fill anatomical defects; its architecture can be designed to provide the mechanical properties necessary to support cell growth, proliferation, differentiation, and motility; and it can be engineered to provide growth factors, drugs, therapeutics, and genes to stimulate tissue regeneration. An inherent property of nanofibers is that they mimic the extracellular matrices (ECM) of tissues and organs. The ECM is a complex composite of fibrous proteins such as collagen and fibronectin, glycoproteins, proteoglycans, soluble proteins such as growth factors, and other bioactive molecules that support cell adhesion and growth. Studies of cell-nanofiber interactions have shown that cells adhere and proliferate well when cultured on polymer nanofibers27, 28, 29.
One of our aims is to fabricate electrospun polymer nanofiber scaffolds for engineering blood vessels, nerves, skin, and bone. We have demonstrated that human coronary artery smooth muscle cells cultured on synthetic nanofibrous scaffolds of the copolymer poly(L-lactic acid)/poly(ɛ-caprolactone), or PLLA/PCL, show normal morphology and good proliferation. The cells organize along the aligned nanofibers in a directional manner typified by the orientation of the cytoskeletal protein α-actin, suggesting that nanofiber orientation can impart a functional development on the cells30.
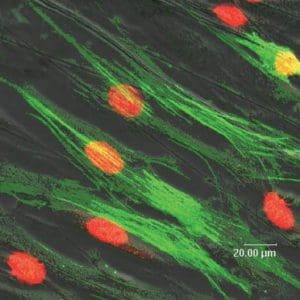
Human coronary artery smooth muscle cells cultured on aligned nanofibers that have been stained for α-actin filaments. (Reprinted with permission from17.
On collagen-modified nanofibers, human coronary artery endothelial cells exhibit cobble-stone morphology, typical of endothelial cells cultured on a polystyrene surface with comparable adhesion and proliferation rates31. On aligned PLLA nanofibers, c17.2 neural cells adhere, elongate along the fibers, and neurites extend along the direction of the aligned fibers (Fig. 9b)32. Human dermal fibroblasts have been demonstrated to grow better on collagen nanofibrous scaffolds than polystyrene tissue culture surfaces (Fig. 9c)33.
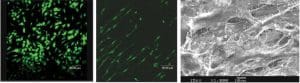
(a) Metabolic dye CMFDA staining of human coronary endothelial cells cultured on random, collagen-blended nanofibers. (b) Metabolic dye CMFDA staining of c17.2 neural cells cultured on aligned nanofibers. (Reprinted with permission from32. © 2005 Elsevier.) (c) Scanning electron micrograph of human fibroblasts cultured on random, pure collagen nanofibers. Metabolic dyes are cell stains that only fluoresce or produce a color in live cells.
A recent study carried out with human coronary endothelial cells cultured on nanofibrous scaffolds34 indicates that nanofiber scaffolds positively promote cell-matrix and cell-cell interactions, with the cells having a normal phenotypic shape and gene expression. This can be attributed to the ECM-like properties of the nanofiber scaffolds that mimic the natural tissue environment.
Further research is required to elucidate the influence of nanofibers on the biochemical pathways and cellular signaling mechanisms that regulate cell morphology, growth, proliferation, differentiation, motility, and genotype. Insight into how natural ECM components secreted by cells replace the biodegradable polymeric scaffolds is also needed. This complete understanding of cell-nanofiber scaffold interactions will pave the way for successful engineering of various tissues and organs, such as vascular grafts, nerve, skin and bone regeneration, cornea transplants, skeletal and cardiac muscle engineering, gastrointestinal and renal/urinary replacement therapy, and even stem cell expansion and differentiation to specific cells types and organ regeneration.
In the pharmaceutical and cosmetic industry, nanofibers are promising tools for controlled delivery of drugs, therapeutics, molecular medicines, and body-care supplements. For example, DNA covalently attached to a patterned carbon nanofiber array and inserted into cells by centrifuging the cells onto the array, does not affect cell viability and the gene encoded by the inserted DNA is expressed35. This could pave the way for the development of a ‘smart’ polymeric drug delivery system.
In another system36, 37, a drug-bound, pH-responsive polymer is targeted to diseased cells through cell receptor binding of a ligand. It is subsequently endocytosed into the endosomal compartment of the cells. In the low pH environment of the endosome, the polymer backbone separates from the drug, destabilizes the endosomal membrane, and releases the drug into the cytoplasmic compartment of the cells. This system of drug delivery can also be used to deliver therapeutics, silencing RNA, antisense oligonucleotides, and vaccines to specific cell types, targeting specific compartments and organelles.
The core-shell nanofibers developed in our laboratory can also be used to encapsulate drugs and therapeutics for drug delivery applications. Release kinetic studies of core-shell nanofibers with fluorescein-isothiocyanate-conjugated bovine serum albumin (FITC-BSA) encapsulated in the core show a gradual release of FITC-BSA when cultured with human dermal fibroblasts (HDFs), instead of a burst release profile without the cells. However, a faster release of FITC-BSA is observed when the nanofibers are cultured with cells. This is likely to be the result of a higher polymer degradation rate in the presence of degradative enzymes secreted by the cells, which could be desirable for applications like wound dressing, where an initially higher but sustained release of antibiotics is preferred. This release profile is crucial for regulating cell growth if the nanofiber scaffolds for tissue engineering applications are to encapsulate bioactive molecules or allow slow passive delivery if the nanofibers are used for drug delivery applications. Biological or chemical ligands can be conjugated onto the nanofibers for cell-specific targeting, or as biosensors responsive to physiological changes to mediate controlled delivery of insulin in diabetes patients. In cosmetics, nanofiber masks can be impregnated with skin-revitalizing factors for skin health and renewal.
Nanofibers can also be fabricated from shape-memory materials38. These can be implanted into the body using a laparoscope (a long, slender medical instrument for examining the interior of an organ or to perform minor surgery), minimizing complex surgical procedures. The fibers then change shape in response to the increased temperature. Such nanofibers have potential as vascular stents, bone void fillers39, hernia repairs, and general tissue repairs40. Because of the large surface-to-volume ratio, nanofibers can also be used in diagnostics for large-scale disease and genetic screening and even as filters in medical instruments for membrane-impermeable biomolecules, bacteria, and viral particles.
Biotechnology and environmental engineering applications
High porosity, interconnectivity, microscale interstitial space, and a large surface-to-volume ratio mean that nonwoven electrospun nanofiber meshes are an excellent material for membrane preparation, especially in biotechnology and environmental engineering applications. Ligand molecules, biomacromolecules, or even cells can be attached or hybridized with the nanofiber membrane for applications in protein purification and waste water treatment (affinity membranes), enzymatic catalysis or synthesis (membrane bioreactors), and, in the future, chemical analysis and diagnostics (biosensors).
Electrospun nanofibers can form an effective size exclusion membrane for particulate removal from wastewater. Particle removal from air by a nanofiber membrane has been studied by Gibson et al.41. The nanofiber membrane shows an extremely effective removal (∼100% rejection) of airborne particles with diameters between 1 μm and 5 μm by both physical trapping and adsorption. For particle removal from aqueous solution, our recent results show that electrospun membranes can successfully remove particles 3-10 μm in size (>95 % rejection) without a significant drop in flux performance42. No particles were found trapped in the membrane, so the membrane could be effectively recovered upon cleaning. This opens up new avenues of application of electrospun membranes for the pretreatment of water prior to reverse osmosis.
In our laboratory, nanofiber membranes are also being tested as affinity (or adsorptive) membranes. Affinity membranes are a broad class of membranes that selectively capture specific target molecules (or ligates) by immobilizing a specific capturing agent (or ligand) onto the membrane surface. In biotechnology, affinity membranes have applications in protein (such as IgG) purification and toxin (such as endotoxin) removal from bioproducts. In the environmental industry, affinity membranes have applications in organic waste removal and heavy metal removal in water treatment.
To be used as affinity membranes, electrospun nanofibers must be surface functionalized with ligands. In most cases, the ligand molecules should be covalently attached on the membrane to prevent leaching of the ligands. Cellulose nanofiber membranes have been surface functionalized with cibacron blue for the purification of albumin43. Cellulose nanofiber membranes functionalized with protein A/G (a recombinant 50 449 Da protein from Pierce Biotechnology that has an increased ability to bind IgG molecules) shows a high ability to capture IgG molecules with a capacity of ∼134 μg/cm2, which is higher than that of the commercialized membrane (∼80 μg/cm2).
Water pollution is now becoming a critical global issue. One important class of inorganic pollutant of great physiological significance is heavy metals, e.g. Hg, Pb, Cu, and Cd. The distribution of these metals in the environment is mainly attributed to the release of metal-containing wastewaters from industries. For example, copper smelters may release high quantities of Cd, one of the most mobile and toxic among the trace elements, into nearby waterways44. It is impossible to eliminate some classes of environmental contaminants completely, such as metals, by conventional water purification methods. Affinity membranes will play a critical role in wastewater treatment to remove (or recycle) heavy metals ions in the future. Polymer nanofibers functionalized with a ceramic nanomaterial, such as hydrated alumina/alumina hydroxide and iron oxides, could be suitable materials for fabrication of affinity membranes for water industry applications. The polymer nanofiber membrane acts as a carrier of the reactive nanomaterial that can attract toxic heavy metal ions, such as As, Cr, and Pb, by adsorption/chemisorption and electrostatic attraction mechanisms.
Compared with heavy metal pollutants, overall water quality is much more sensitive to organic pollutants. Although such organics are usually no more than 1% of the pollution in a river, they tend to use up its dissolved oxygen, making the water unable to sustain life. While the transformations and pathways of metals in the environment have been studied to some extent, much less information is available on most commercial organic products because of their complex structures. Again, affinity membranes provide an alternative approach for removing organic molecules from wastewater. For example, β-cyclodextrin is a cyclic oligosaccharide comprising of seven glucose units. It has a stereo-specific toroidal structure with a hydrophobic interior and hydrophilic exterior that can capture hydrophobic organic molecules from water by forming an inclusion complex. β-cyclodextrin has been introduced into a poly(methyl methacrylate) nanofiber membrane using a physical mixing method to develop an affinity membrane for organic waste removal45.
Electrospun nanofibers have also received great attention for sensor applications because of their unique high surface area. This is one of the most desirable properties for improving the sensitivity of conductometric sensors because a larger surface area will absorb more of a gas analyte and change the sensor’s conductivity more significantly. Nanofibers functionalized with a semiconductor oxide such as MoO3, SnO2, or TiO2 show an electrical resistance that is sensitive to harmful chemical gases like ammonia and nitroxide46. Single polypyrrole nanofibers containing avidin were studied as biosensors for detecting biotin-labeled biomolecules such as DNA. Specific binding of the biomolecules to the nanofibers changes the electrical resistance of a single nanofiber47. A fluorescent polymer, poly(acrylic acid)-poly(pyrene methanol), or PAA-PM, was used as a sensing material for the detection of organic and inorganic waste. The fluorescence is quenched by adsorbed metal ions Fe3+ or Hg2+ or 2,4-dinitrotoluene (DNT) on the nanofiber surfaces48. In our laboratory, nylon-6 nanofiber was functionalized with biotinylated glucose oxidase to develop a novel biosensor for testing glucose concentration49.
Defense and security applications
Military, firefighter, law enforcement, and medical personnel require high-level protection when dealing with chemical and biological threats (which include chemicals like nerve agents, mustard gas, blood agents such as cyanides, and biological toxins such as bacterial spores, viruses, and rickettsiae) in many environments ranging from combat to urban, agricultural, and industrial. Current protective clothing is based on full barrier protection such as hazardous materials (HAZMAT) suits, or permeable adsorptive protective overgarments such as those used by the US military. The obvious limitations of these suits are weight and moisture retention, which prevent the user from donning them for long periods.
Nanostructures with their small size, large surface area50, and light weight will improve, by orders of magnitude, our capability to:
- Detect chemical and biological warfare agents with sensitivity and selectivity;
- Protect through filtration and destructive decomposition of harmful toxins; and
- Provide site-specific in vivo prophylaxis.
Polymer nanofibers are considered as excellent membrane materials for this purpose owing to their light weight, high surface area, and breathable (porous) nature51. The high sensitivity of nanofibers toward warfare agents makes them excellent candidates as sensing interfaces for chemical and biological toxins in concentration levels of parts per billion52. Governments across the world are investing in strengthening the protection levels offered to soldiers in the battlefield53. Various methods of modifying nanofiber surfaces to enhance their capture and decontamination capability of warfare agents are currently under investigation. One protection method is through chemical surface modification and attachment of reactive groups such as oximes, cyclodextrins, and chloramines54, 55 that bind and detoxify warfare agents.
In association with the Defense Science and Technology Agency (DSTA) in Singapore, our laboratory is working on functionalizing nanofibers to be used in facemasks for chemical and biowarfare defense. The facemask consists of two main components: a high-efficiency particulate air (HEPA) filtering layer and an activated charcoal bed that adsorbs harmful gases and contaminants.
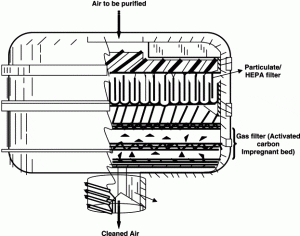
Schematic showing the cross section of a facemask canister used for protection from chemical and biological warfare agents.
Nanofiber membranes may be used to replace the activated charcoal in adsorbing toxins from the atmosphere. Active reagents can be embedded into the nanofiber membrane by chemical functionalization, post-spinning modification, or through using nanoparticle polymer composites. Preliminary tests using chemical warfare simulators such as paraoxon and dimethyl methyl phosphonate on the functionalized fibers show evidence of decontamination. Metal nanoparticles (Ag, MgO, Ni, Ti, etc.), which have proven abilities in decomposing warfare agents, can also be embedded in the nanofibers.
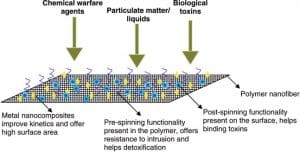
Schematic of the incorporation of functional groups into a polymer nanofiber mesh.
There are many avenues for future research in nanofibers from the defense perspective. As well as serving protection and decontamination functions, nanofiber membranes will also have to provide the durability, washability, resistance to intrusion of all liquids, and tear strength required of battledress fabrics.
Energy generation applications:
Natural energy resources such as crude oil, coal, natural gas, and uranium are a necessity for everyday life. Rapid economic growth in Asia and the subsequent increase in demand for energy mean that the rate of oil production is no longer adequate. This is evident in the soaring price of crude oil, which has reached over $60 per barrel56. Large volumes of carbon dioxide emitted by the burning of fossil fuels is also the main culprit in climate change. Thus, there is an urgent need to identify new sources of energy that are environmentally friendly and able to replace current supplies. Polymer batteries, fuel cells, photovoltaic cells, wind power generators, and geothermal power generators are some possible alternatives.
Given their high porosity and inherent large total surface area, electrospun nanofiber membranes are being considered for polymer batteries57, 58, 59, photovoltaic cells60, 61, 62, 63, and polymer electrolyte membrane fuel cells (PEMFCs).
Polymer batteries have been developed for PC notebooks and cell phones to replace conventional, bulky lithium batteries. The components of polymer batteries are a carbon anode, a lithium cobalt oxide cathode, and a polymer gel electrolyte. When a battery is subjected to charging, Li+ ions are confined in the carbon anode. On discharging, the Li+ ions move to cathode. Noteworthy properties of polymer batteries are less electrolyte leakage, high dimension flexibility, and high energy density per weight. However, there is still a need to improve energy density per weight of polymer batteries to increase their market share. Choi et al.57 and Kim et al.59 have assembled a new type of polymer battery using poly(vinylidene fluoride), or PVDF, nanofiber membranes. The porous structure of the PVDF nanofiber membrane favors high uptake (350 wt.%) of lithium electrolyte so that electrolyte leakage is reduced. These factors make it possible to hold a large quantity of lithium electrolyte in thinner battery packs. The large surface area of the nanofibrous network also enhances ion conductivity, thus polymer batteries comprising nanofiber membranes may improve energy density per weight as compared with conventional polymer batteries.
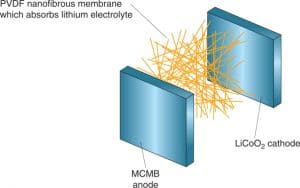
Polymer battery assembled by sandwiching PVDF nanofiber membranes between a mesocarbon microbead (MCMB) anode and a LiCoO2 cathode58, 59.
Most conventional photovoltaic cells use single-crystalline, polycrystalline, or amorphous Si. It is well known that a single-crystal Si cell can achieve an energy translation efficiency of ∼20%, and this value is higher than other types of solar cells. However, the biggest shortcoming for single-crystal Si solar cells is their high manufacturing cost. There is also a need for a large surface area to obtain sufficient electrical output.
As an alternative, Grätzel and colleagues64 have developed dye-sensitized solar cells. The principle here is that sensitizing dye molecules coated onto TiO2 nanoparticles absorb photons and transfer excited electrons through the conduction band of TiO2 to the cathode. A nanotopographic TiO2 layer works as the electrode and enhances the total surface area to achieve a high electrical output. Dye-sensitized solar cells are less costly to manufacture than Si-based solar cells, but there are issues that need to be addressed, including reducing electrolyte leakage and improving the energy conversion efficiency (generally ∼4–10%). With respect to electrolyte leakage, an alternative solution is to use a viscous polymer gel electrolyte. However, it is difficult to infuse a viscous gel into a conventional TiO2 nanotopographic layer. Song et al.61, 62, 63 have solved this problem by using TiO2 nanofiber membranes fabricated by electrospinning in combination with sol-gel processes (Fig. 15). The viscous polymer gel electrolyte can easily penetrate into the porous nanofiber membrane. Their assembled TiO2 nanofiber dye-sensitized solar cells are able to achieve an energy conversion efficiency of 6.2%63.
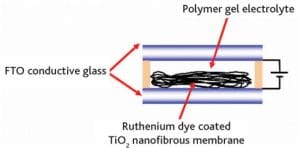
Dye-sensitized solar cells assembled using TiO2 nanofiber membranes61, 62, 63.
Electricity generation in PEMFCs is through the chemical reaction of hydrogen at the anode and oxygen at the cathode. Protons are transmitted through an electrolyte membrane that contains distilled water, while electrons are transmitted from the anode to the cathode. The key properties of electrolyte membranes are high proton conductivity and shielding of electron transport. As the membrane needs to hold distilled water for proton conductivity, water retention of the membrane is also important. Nafion® (DuPont), a perfluorosulfonic acid polymer film, has been widely used so far. However, Nafion membranes are expensive at up to $800/kg. For the same membrane area, electrospun Nafion fiber membranes require less material than conventional Nafion fuel cell membranes, thereby reducing cost. Porous nanofiber membranes are also able to hold distilled water, thus enhancing proton conductivity. Therefore, such nanofiber membranes have the potential to be used in PEMFCs.
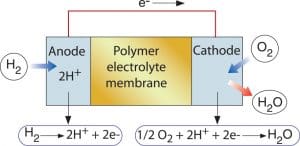
Principle of electricity generation in fuel cells.
Conclusion:
Given the versatility of electrospinning for generating highly porous nanofiber meshes made out of different materials, it is no surprise that it has found possible uses in different fields ranging from healthcare, biotechnology, and environmental engineering to defense and security, and energy generation. Electrospinning may be able to produce microengineered scaffolds for tissue engineering. Improved wound dressings could be made out of nanofiber meshes impregnated with drugs. Membranes for water treatment or use in biotechnology could be made of electrospun fibers. Nanofiber clothing and filters could deal more effectively with chemical and biological threats. In the future, we may no longer be dependent on crude oil thanks to more efficient conversion of other energy sources to electricity. With the ability to mass-produce nanofibers, electrospinning may well be one of the most significant nanotechnologies of this century.